Exciton
Introduction
An exciton is a bound state of an electron and an electron hole which are attracted to each other by the electrostatic Coulomb force. It is a quasi-particle that exists in insulators, semiconductors, and some liquids. The concept of the exciton was first proposed by Yakov Frenkel in 1931 when he described the phenomenon in molecular crystals. Excitons play a crucial role in the optical properties of materials and are fundamental in the study of optoelectronic devices, such as solar cells, light-emitting diodes (LEDs), and lasers.
Types of Excitons
Excitons can be classified into several types based on their binding energy, spatial distribution, and the nature of the materials in which they are found.
Frenkel Excitons
Frenkel excitons are typically found in organic molecular crystals and some inorganic materials. They are characterized by a small radius, often on the order of a few angstroms, and a high binding energy. The electron and hole are tightly bound and localized on the same molecule or atom. Frenkel excitons are significant in organic semiconductors and are responsible for the optical properties of many organic materials.
Wannier-Mott Excitons
Wannier-Mott excitons are found in inorganic semiconductors and have a much larger radius compared to Frenkel excitons. Their binding energy is relatively low, and they can extend over several lattice constants. The electron and hole are less tightly bound and can move more freely within the crystal lattice. Wannier-Mott excitons are essential in the study of semiconductor physics and are crucial for understanding the behavior of materials like silicon, gallium arsenide, and other semiconductor compounds.
Charge-Transfer Excitons
Charge-transfer excitons occur in materials where the electron and hole are located on different molecules or atoms. These excitons are common in organic photovoltaic materials and play a vital role in the charge separation process in solar cells. The spatial separation of the electron and hole can lead to a longer-lived exciton, which is beneficial for the efficiency of photovoltaic devices.
Surface Excitons
Surface excitons are found at the interfaces of materials, such as the surface of a semiconductor or the boundary between two different materials. These excitons are influenced by the properties of the surface or interface and can exhibit unique behaviors compared to bulk excitons. Surface excitons are important in the study of nanostructures and thin films, where surface effects can dominate the material's properties.
Exciton Dynamics
The behavior of excitons in a material is governed by several factors, including their formation, recombination, and diffusion.
Formation
Excitons are typically formed when a material absorbs a photon with energy greater than its bandgap. This absorption process excites an electron from the valence band to the conduction band, leaving behind a hole. The electron and hole can then bind together to form an exciton. The efficiency of exciton formation depends on the material's electronic structure and the nature of the photon absorption process.
Recombination
Excitons can recombine through radiative or non-radiative processes. In radiative recombination, the electron and hole annihilate, emitting a photon. This process is the basis for light emission in LEDs and lasers. Non-radiative recombination involves the transfer of energy to lattice vibrations (phonons) or other excitations, leading to the dissipation of energy as heat. The balance between radiative and non-radiative recombination determines the efficiency of optoelectronic devices.
Diffusion
Excitons can move through a material by diffusion, which is influenced by their binding energy and the material's properties. In organic materials, exciton diffusion is often limited by the small radius and high binding energy of Frenkel excitons. In inorganic semiconductors, Wannier-Mott excitons can diffuse over larger distances due to their lower binding energy and larger radius. Exciton diffusion is a critical factor in the design of devices like solar cells, where efficient charge separation and transport are essential.
Exciton-Phonon Interaction
Excitons interact with lattice vibrations, or phonons, in a material. This interaction can influence the exciton's properties and behavior.
Phonon-Assisted Absorption and Emission
In some materials, exciton formation and recombination can be assisted by phonons. Phonon-assisted absorption occurs when a photon with energy less than the bandgap is absorbed, with the additional energy provided by a phonon. Similarly, phonon-assisted emission involves the recombination of an exciton with the simultaneous emission of a photon and a phonon. These processes are important in indirect bandgap semiconductors, where direct photon absorption and emission are less likely.
Exciton-Phonon Scattering
Excitons can scatter off phonons, leading to changes in their momentum and energy. This scattering process can affect exciton diffusion and recombination rates. In some cases, strong exciton-phonon coupling can lead to the formation of polarons, where the exciton is dressed by a cloud of phonons. Polaron formation can significantly alter the exciton's properties and is an important consideration in the study of exciton dynamics.
Exciton-Exciton Interaction
In materials with high exciton densities, exciton-exciton interactions can become significant. These interactions can lead to various phenomena, such as exciton-exciton annihilation, where two excitons collide and recombine non-radiatively. Exciton-exciton interactions can also lead to the formation of biexcitons, which are bound states of two excitons. Biexcitons have unique properties and can be studied using techniques like two-photon absorption spectroscopy.
Excitonic Effects in Optoelectronic Devices
Excitons play a crucial role in the operation of various optoelectronic devices.
Solar Cells
In organic and hybrid solar cells, excitons are generated upon photon absorption and must be efficiently separated into free charge carriers to generate electricity. The design of these devices often involves the use of donor-acceptor heterojunctions, where the exciton dissociates at the interface between two materials with different electron affinities. The efficiency of exciton dissociation and charge transport is critical for the performance of solar cells.
Light-Emitting Diodes (LEDs)
In LEDs, excitons are formed by the injection of electrons and holes into the active layer of the device. The radiative recombination of excitons results in light emission. The efficiency of LEDs depends on the balance between radiative and non-radiative recombination processes. Materials with high exciton binding energies and efficient radiative recombination are preferred for LED applications.
Lasers
Excitons can also be used to achieve population inversion and stimulated emission in semiconductor lasers. In these devices, excitons are generated by optical or electrical pumping, and their radiative recombination leads to the emission of coherent light. The design of excitonic lasers involves optimizing the material properties to achieve high exciton densities and efficient light emission.
Excitons in Low-Dimensional Systems
Excitons exhibit unique properties in low-dimensional systems, such as quantum wells, quantum dots, and two-dimensional materials.
Quantum Wells
In quantum wells, excitons are confined in one dimension, leading to enhanced binding energies and altered optical properties. The confinement can result in discrete exciton energy levels, which can be probed using techniques like photoluminescence spectroscopy. Quantum wells are used in various optoelectronic devices, including lasers and photodetectors.
Quantum Dots
Quantum dots are zero-dimensional systems where excitons are confined in all three dimensions. This confinement leads to even higher binding energies and size-dependent optical properties. Quantum dots can emit light with tunable wavelengths, making them useful for applications in displays, bioimaging, and quantum computing.
Two-Dimensional Materials
Two-dimensional materials, such as transition metal dichalcogenides (TMDs), exhibit strong excitonic effects due to reduced dielectric screening and enhanced Coulomb interactions. Excitons in these materials have high binding energies and can form complex states like trions (charged excitons) and biexcitons. The study of excitons in two-dimensional materials is an active area of research with potential applications in next-generation optoelectronic devices.
Experimental Techniques for Studying Excitons
Several experimental techniques are used to study excitons and their properties.
Photoluminescence Spectroscopy
Photoluminescence spectroscopy involves the excitation of a material with a light source and the measurement of the emitted light. This technique is used to probe the energy levels and recombination dynamics of excitons. Photoluminescence spectra can provide information about exciton binding energies, lifetimes, and interactions with other excitations.
Absorption Spectroscopy
Absorption spectroscopy measures the absorption of light by a material as a function of wavelength. This technique can be used to determine the energy levels of excitons and their binding energies. Absorption spectra can also reveal information about phonon-assisted processes and exciton-phonon interactions.
Time-Resolved Spectroscopy
Time-resolved spectroscopy involves the measurement of the temporal dynamics of excitons following excitation. Techniques like time-resolved photoluminescence and pump-probe spectroscopy can provide insights into exciton formation, recombination, and diffusion processes. Time-resolved measurements are essential for understanding the ultrafast dynamics of excitons in materials.
Two-Photon Absorption Spectroscopy
Two-photon absorption spectroscopy is used to study biexcitons and other multi-exciton states. In this technique, two photons are simultaneously absorbed by a material, leading to the formation of a biexciton. The resulting spectra can provide information about the binding energies and interactions of biexcitons.
Theoretical Models of Excitons
Several theoretical models have been developed to describe excitons and their properties.
Effective Mass Approximation
The effective mass approximation is commonly used to describe Wannier-Mott excitons in semiconductors. In this model, the electron and hole are treated as particles with effective masses that account for the influence of the crystal lattice. The exciton binding energy and radius can be calculated using the Schrödinger equation with a Coulomb potential.
Tight-Binding Model
The tight-binding model is often used to describe Frenkel excitons in molecular crystals. In this model, the electronic states are localized on individual molecules or atoms, and the exciton is described by a wavefunction that is a linear combination of these localized states. The tight-binding model can be used to calculate exciton energy levels and their dependence on the crystal structure.
Bethe-Salpeter Equation
The Bethe-Salpeter equation is a more advanced theoretical approach that accounts for many-body interactions in the description of excitons. This equation is derived from the Green's function formalism and can be used to calculate exciton binding energies, wavefunctions, and optical spectra. The Bethe-Salpeter equation is particularly useful for studying excitons in complex materials and low-dimensional systems.
Applications of Excitons
Excitons have a wide range of applications in various fields of science and technology.
Photovoltaics
Excitons are crucial in the operation of organic and hybrid solar cells. The efficient generation, separation, and transport of excitons are essential for the performance of these devices. Research in excitonics aims to develop new materials and device architectures to improve the efficiency of solar cells.
Light-Emitting Devices
Excitons are responsible for light emission in LEDs and other light-emitting devices. The design of materials with high exciton binding energies and efficient radiative recombination is key to developing high-performance LEDs. Excitonic materials are also being explored for applications in displays and lighting.
Quantum Computing
Excitons in quantum dots and other nanostructures are being investigated for applications in quantum computing. The discrete energy levels and tunable properties of excitons make them suitable for use as qubits in quantum information processing. Research in this area focuses on developing techniques for the coherent control and manipulation of excitons.
Sensing and Imaging
Excitonic materials are used in various sensing and imaging applications. For example, quantum dots are used as fluorescent probes in bioimaging due to their bright and tunable emission. Excitonic sensors can also be developed for detecting environmental changes, chemical species, and biological molecules.
Future Directions in Exciton Research
The study of excitons is a rapidly evolving field with many exciting future directions.
Excitonic Devices
The development of new excitonic devices, such as excitonic transistors and excitonic circuits, is an area of active research. These devices aim to exploit the unique properties of excitons for applications in electronics and photonics. The integration of excitonic devices with existing technologies could lead to new functionalities and improved performance.
Two-Dimensional Materials
The exploration of excitonic effects in two-dimensional materials is a promising area of research. The unique properties of these materials, such as strong Coulomb interactions and reduced dielectric screening, lead to novel excitonic phenomena. The study of excitons in two-dimensional materials could lead to new optoelectronic devices with enhanced performance.
Excitons in strongly correlated systems, such as transition metal oxides and high-temperature superconductors, are an emerging area of interest. The interplay between excitonic effects and other many-body interactions in these materials can lead to new phases of matter and exotic excitations. Understanding these phenomena could provide insights into fundamental physics and lead to new technological applications.
Conclusion
Excitons are fundamental quasi-particles that play a crucial role in the optical and electronic properties of materials. Their study encompasses a wide range of disciplines, including condensed matter physics, materials science, and optoelectronics. Advances in the understanding and manipulation of excitons have the potential to drive innovations in various technologies, from solar cells and LEDs to quantum computing and sensing. As research in this field continues to evolve, excitons will remain a central focus in the quest to develop new materials and devices with enhanced performance and novel functionalities.
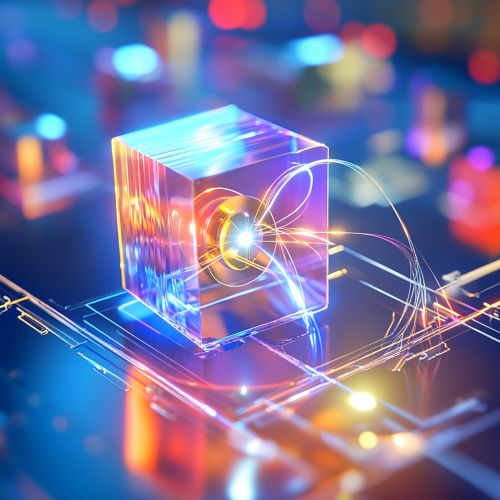
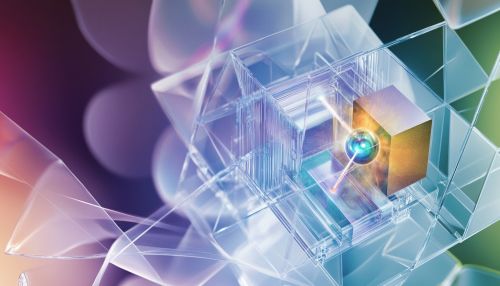