Quantum hardware: Difference between revisions
(Created page with "== Introduction == Quantum hardware refers to the physical systems and devices that are used to implement quantum computing and quantum information processing. Unlike classical computing hardware, which relies on bits to represent information as 0s and 1s, quantum hardware uses quantum bits or qubits, which can exist in multiple states simultaneously due to the principles of quantum superposition and quantum entanglement. This article delves into the...") |
No edit summary |
||
Line 13: | Line 13: | ||
Superconducting qubits are typically cooled to millikelvin temperatures using dilution refrigerators to maintain their quantum coherence. | Superconducting qubits are typically cooled to millikelvin temperatures using dilution refrigerators to maintain their quantum coherence. | ||
[[Image:Detail-92819.jpg|thumb|center|Close-up view of superconducting qubits on a chip.|class=only_on_mobile]] | |||
[[Image:Detail-92820.jpg|thumb|center|Close-up view of superconducting qubits on a chip.|class=only_on_desktop]] | |||
=== Trapped Ion Qubits === | === Trapped Ion Qubits === |
Latest revision as of 13:41, 21 June 2024
Introduction
Quantum hardware refers to the physical systems and devices that are used to implement quantum computing and quantum information processing. Unlike classical computing hardware, which relies on bits to represent information as 0s and 1s, quantum hardware uses quantum bits or qubits, which can exist in multiple states simultaneously due to the principles of quantum superposition and quantum entanglement. This article delves into the various types of quantum hardware, their underlying principles, and the current state of research and development in the field.
Types of Quantum Hardware
Quantum hardware can be categorized based on the physical systems used to realize qubits. The most prominent types include:
Superconducting Qubits
Superconducting qubits are one of the most advanced and widely researched types of quantum hardware. These qubits are created using superconducting circuits that exhibit quantum mechanical properties at very low temperatures. The two primary types of superconducting qubits are:
- **Transmon Qubits**: These qubits are designed to reduce sensitivity to charge noise, making them more stable and easier to control.
- **Flux Qubits**: These qubits utilize the magnetic flux through a superconducting loop to represent quantum states.
Superconducting qubits are typically cooled to millikelvin temperatures using dilution refrigerators to maintain their quantum coherence.
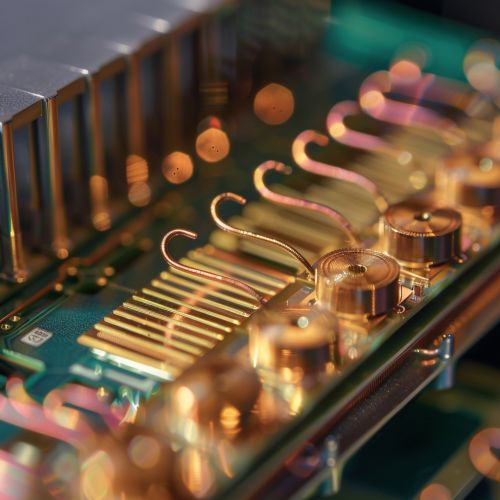
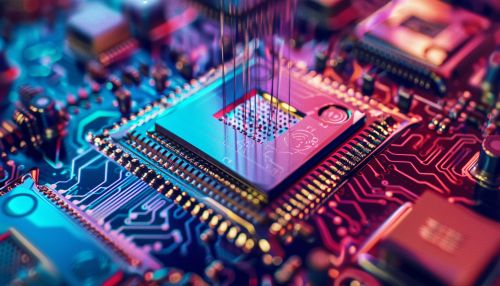
Trapped Ion Qubits
Trapped ion qubits use individual ions confined in electromagnetic traps. These ions are manipulated using laser beams to perform quantum operations. The advantages of trapped ion qubits include long coherence times and high-fidelity gate operations. However, scaling up the number of qubits remains a significant challenge.
Topological Qubits
Topological qubits are based on the principles of topological quantum computing, where information is stored in the global properties of the system rather than the local properties. This makes topological qubits inherently resistant to local errors, providing a robust platform for quantum computing. Majorana fermions are a promising candidate for realizing topological qubits.
Photonic Qubits
Photonic qubits use the quantum states of photons to encode information. These qubits can be manipulated using linear optical elements such as beam splitters and phase shifters. Photonic qubits are advantageous for quantum communication due to their ability to travel long distances with minimal loss.
Neutral Atom Qubits
Neutral atom qubits use individual neutral atoms trapped in optical lattices or tweezers. These qubits are manipulated using laser pulses to perform quantum operations. Neutral atom qubits offer the potential for large-scale quantum computing due to their scalability and long coherence times.
Quantum Gates and Operations
Quantum gates are the fundamental building blocks of quantum circuits, analogous to classical logic gates. They manipulate the state of qubits to perform quantum computations. Some of the most common quantum gates include:
- **Pauli Gates (X, Y, Z)**: These gates perform rotations around the X, Y, and Z axes of the Bloch sphere.
- **Hadamard Gate (H)**: This gate creates superposition states by transforming the basis states |0⟩ and |1⟩ into equal superpositions.
- **CNOT Gate**: The controlled-NOT gate flips the state of a target qubit based on the state of a control qubit, enabling entanglement between qubits.
- **Toffoli Gate**: Also known as the controlled-controlled-NOT gate, it is a universal gate for reversible classical computation.
Quantum Error Correction
Quantum error correction is essential for maintaining the coherence of qubits in the presence of noise and decoherence. Quantum error-correcting codes, such as the Shor code and the Surface code, are used to detect and correct errors without measuring the quantum state directly. These codes rely on the principles of quantum redundancy and syndrome measurement to protect quantum information.
Quantum Hardware Challenges
Despite significant progress, several challenges remain in the development of practical quantum hardware:
- **Scalability**: Scaling up the number of qubits while maintaining coherence and control is a major hurdle.
- **Decoherence**: Quantum systems are highly susceptible to environmental noise, leading to loss of coherence.
- **Error Rates**: Reducing gate error rates to achieve fault-tolerant quantum computation is critical.
- **Interconnects**: Efficiently interconnecting qubits to perform complex quantum operations is a technical challenge.
Current Research and Development
Research in quantum hardware is a rapidly evolving field, with numerous academic and industrial efforts aimed at overcoming existing challenges. Some notable developments include:
- **Quantum Supremacy**: Achieving quantum supremacy, where a quantum computer outperforms the best classical computers, has been a significant milestone. Google's demonstration of quantum supremacy using a superconducting qubit processor is a notable example.
- **Quantum Volume**: IBM introduced the concept of quantum volume as a metric to measure the performance of quantum computers, taking into account factors such as qubit count, connectivity, and error rates.
- **Hybrid Quantum-Classical Systems**: Combining quantum and classical computing resources to solve complex problems more efficiently is an area of active research.
Future Directions
The future of quantum hardware research is focused on several key areas:
- **Fault-Tolerant Quantum Computing**: Developing error-corrected quantum systems that can perform reliable computations over extended periods.
- **Quantum Networking**: Creating quantum networks to enable secure communication and distributed quantum computing.
- **Quantum Algorithms**: Designing new quantum algorithms that can leverage the unique capabilities of quantum hardware to solve real-world problems.